NUCLEAR MAGNETIC RESONANCE BASICS
Magnetic resonance is
a phenomenon by which a nucleus
of an atom absorbs electromagnetic
radiation of a specific frequency in the presence of a strong
magnetic field. Isidor Isaac Rabi (1898 to 1988), an American
physicist born in Austria, first detected magnetic resonance
in 1938. Since then, magnetic resonance has been applied to
the detection of light atoms (such as hydrogen in
hydrocarbons) and has been used as a nondestructive way to
study the human body.
Laboratory NMR apparatus used for medical diagnosis consists of
a magnet which provides a strong, steady magnetic field that is
as uniform as possible and a coil that produces an oscillating
magnetic field perpendicular to the static field direction. A
relatively small, compact sample, such as a person, is placed inside
the coil. For borehole NMR, the sample - namely the Earth - is not
inside the measurement apparatus, but outside of it. It requires a
leap of imagination to see how NMR measurements can be made
"inside-out" on a sample external to the apparatus.
This design problem has been solved in a number of distinctly
different ways. The Schlumberger CMR apparatus consists of two samarium
cobalt magnets, each about one foot (30 centimeters) long, that
project a magnetic field into the formation. An antenna located in a
cylindrical well on the face of the apparatus generates the
oscillating magnetic field, which is also projected into the rock. The two magnetic fields are substantially perpendicular
within the volume of rock being examined, which extends about an
inch (2.5 cm) into the formation. The antenna is used as both
transmitter and receiver. A durable polymer wear plate covering the
antenna is the only nonmetallic surface on the exterior of the
tool. Metal cannot be used here because it would prevent the
transmission and reception of the magnetic field.
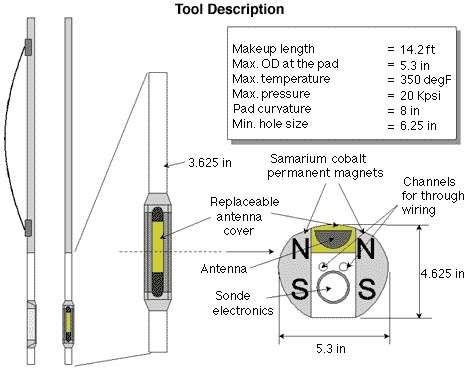
Schlumberger CMR tool design
All modern NMR tools use a
permanent magnet to align the proton spin axis of the reservoir
fluid. Older tools (1960 - 1988+/-) relied on the Earth's magnetic
field as the permanent magnet. This probably accounts for their
lousy performance and unreliable results. All tools use a radio
frequency transmitter - receiver to tip the spin axis, then record
the electromagnetic signal emitted by the protons as they process
back to their original spin direction. The transmitter operates at
the Larmor frequency, which depends on the strength of the permanent
magnet on the tool. On a CMR, for hydrogen, this is 3.8 MHz.
The rate of decay of the emitted
energy is transformed into a moveable fluids measurement, and on
newer tools, a measure of irreducible and clay bound water are also
obtained.
Unlike many other logging tools,
the NMR design and operating principles are somewhat different
between different service suppliers. A Schlumberger CMR tool
investigates a chunk of rock about the size of a good Cuban cigar,
about 1 inch from the borehole wall, in front of the permanent
magnet on the face of the tool. Halliburton's MRIL tool (NUMAR
design) measures a thin cylinder about 2 inches inside the rock,
circling the entire borehole. The Baker Atlas MREX tool sees a 120
degree segment of a cylinder about 2 inches into the rock.
 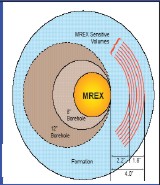
Measured rock volume for MRIL (red circle), CMR (red dot), and MREX
(grey)
NMR OPERATING PRINCIPLES
The following
description of the operating principle for NMR is based on a Schlumberger CMR
tool.
Many (though not
all) atomic nuclei can be thought of as very small bar magnets, with
a north pole and a south pole. The nuclei spin at a constant rate,
with the spin axis exactly coinciding with the line between the
north and south poles.
Spinning bar magnets are pretty common in nature.
Individual iron atoms, the Earth, the Sun, several planets and
neutron stars are all spinning bar magnets. The Earth is a more
complicated spinning bar magnet than an atomic nucleus, because the
geographical north pole (spin axis) of the Earth does not
coincide with its magnetic north pole. Nuclei are better behaved:
their magnetic and geographical poles coincide exactly.
The hydrogen nucleus, which consists of a single proton, is
magnetic and an abundant component of water, gas, and oil.
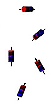 Ordinarily, the
north poles of nuclei point in random direction, but many are
roughly aligned with the Earth's magnetic field.
The first step of a modern NMR measurement is to align the nuclear
magnets with a strong magnetic field. This makes them line up, with north poles of the nuclei
pointing to the south pole of the magnet. All the magnets are in a
uniform equilibrium (low energy) state.
The second step is to apply another magnetic field at 90 degrees to
the first, and in
resonance with the nuclear motion.
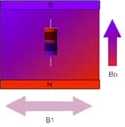 |
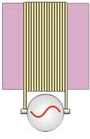 |
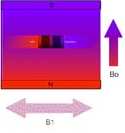 |
An oscillating magnetic field called
B1 is perpendicular to the field of the permanent magnet,
B0. |
|
After a while the nuclei are tipped so
that they are rotating in the plane that is perpendicular to
the B0 field. |
Because the nucleus is spinning, it behaves
like a gyroscope or toy top. When a gyro or top is pointing straight
up in the earth's gravitational field, it just spins. But if it tips
away from vertical it goes into an orbital motion called
precession. The precession speed, which is much slower than the spin
speed, depends on the size and shape of the gyro, its spin speed, and
the strength of gravity.
When a nucleus is tipped away from the direction of the strong
magnetic field, it too precesses. The precession speed depends on
the properties of the nucleus (rate of spin, etc.) and the strength
of the magnetic field — very similar to the gyroscope. These
properties never change, so all we need to know is the strength of
the magnetic field to accurately predict the precession frequency.
That's the frequency we must apply to get the nucleus
tipped away from the main magnetic field and precessing. motion.
Step three is to turn off the
resonant magnetic field. Precession will continue for quite a while. All
we need is a quick burst of radio waves, lasting maybe 10
microseconds to keep them going for as much as
several seconds.
As long as the nuclei are out of
alignment with the big magnet (out of equilibrium) they radiate
radio waves. Part of the NMR equipment
is a radio receiver, to catch the emissions from the nuclei while
they are moving. The first NMR apparatus was built with old World
War II radar sets, which have both a radio transmitter and receiver
in one unit.
The precession of the nucleus will find a
way to slowly return to equilibrium, oriented with the field of the
permanent magnet in the NMR tool.
There are many ways for a nucleus to lose energy and
return to equilibrium. One way, if the nucleus is in a liquid
molecule, like water, is when it hits a solid surface. Every time
the molecule hits a solid surface, the nucleus has a chance to
return to happy alignment along the strong magnetic field. This is
called relaxation.
In larger pores the fluid molecules have more room to move around
without bumping into the walls, so these collisions are less
frequent. In a rock, NMR relaxation depends on the size of the
pores: the larger the pores, the longer the NMR relaxation time.
The sensitivity of NMR to pore size has two simple, but very
powerful, applications. The first is to estimate permeability, which is
determined by the size of its pores. More precisely, the
permeability is proportional to the square of the diameter of the
pores, so one expects the permeability to be proportional to the
square of the NMR relaxation time. We have confirmed this
relationship by doing laboratory tests on hundreds of different
kinds of rocks.
The second
application of NMR data is to determine a distribution of pore
sizes. Since pores within a single rock can vary greatly in size,
the distributions are very broad. The pore size distribution tells
geologists a lot about a rock.
Apart from pore size and pore size
distribution, the chief application of the NMR tool is to determine
moveable fluid volume of a rock. This is the pore space excluding
clay bound water (CBW) and irreducible water (BVI). Neither of these
are moveable in the NMR sense, so these volumes are not easily
observed on older logs. On modern tools both CBW and BVI
can often be seen in the signal response after transforming the
relaxation curve to the porosity domain. Note that some of the
moveable fluids (BVM) in the NMR sense are not actually moveable in
the oilfield sense of the word. Residual oil and gas, heavy oil, and
bitumen may appear moveable to the NMR precession measurement, but
these will not necessarily flow into a well bore.
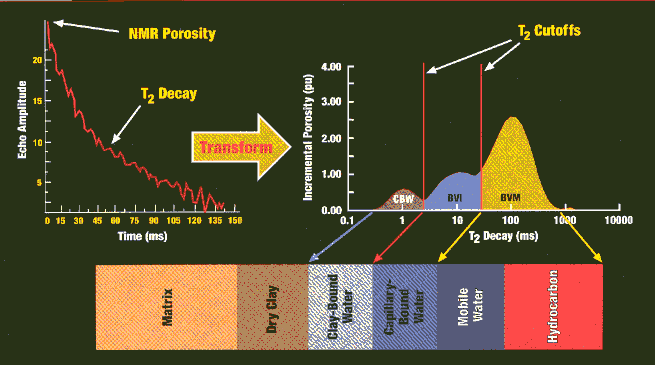
Transforming the precession decay time curve into the porosity
domain, showing breakdown of CBW,
BVI and BVM. The cutoffs vary with lithology.
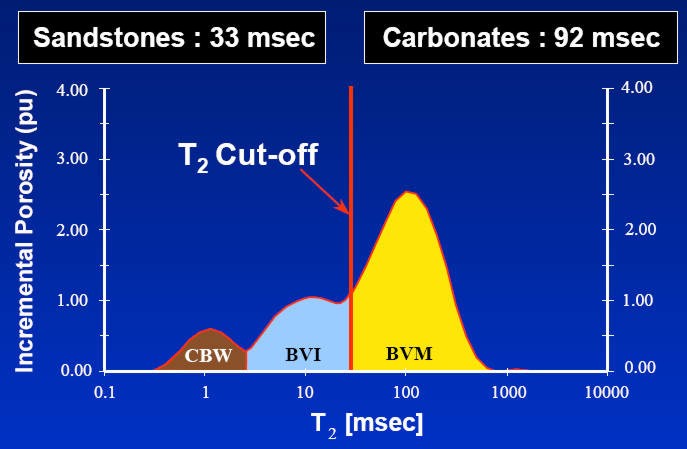
T2 distribution for heavy oil (red curve) and light oil (yellow) -
area under the curves are equal, so total porosity is independent of
hydrocarbon density. T2 cutoff between BVI and BVM varies with
lithology - total porosity does not vary with lithology, but BVI and
BVM do vary with lithology.
The
matrix and dry clay terms of NMR response are zero. As a result
the NMR porosity is said to be independent of lithology.
However, this is only true for total porosity (the total area
under the shaded curve in the above illustration). The boundary
between CBW and BVI, and the boundary between BVI and BVM, do
depend on lithology and may vary foot by foot through the
reservoir. As a result, the choice of fixed T2 cutoff times to
represent these boundaries is not a good idea, and more
elaborate methods are now being used.
An NMR log
run today can display clay bound water (CBW), irreducible water
(capillary bound water, BVI), and mobile fluids (hydrocarbon plus
water, BVM), also called free fluids or free fluid index (FFI).
On older logs, only free fluids (FFI) are recorded and some subtractions,
based on other open hole logs, are required to obtain BVI and
CBW.
|